In search of a parallel universe
What are parallel universes, and why do we think they might exist?

Theoretical physicist Leonardo Senatore from the Kavli Institute for Particle Astrophysics and Cosmology explains.
Want to talk to Leonardo Senatore about parallel universes? Find him at Symmetry's Twitter account at noon Central today!
Have a burning question about particle physics? Let us know via email or Twitter (using the hashtag #AskSymmetry). We might answer you in a future video!
You can watch a playlist of the #AskSymmetry videos here.
A bright idea
Can a biochemistry technique win the battle against background for scientists studying the nature of neutrinos?

While we read, think, move or just perceive the world around us, thousands of neurons fire in our brain. Ions, like little messengers, jump from neuron to neuron and create a cascade of information transfer. Using a technique called single-molecule fluorescence imaging, neuroscientists can make these cascades glow.
David Nygren, a physics professor who studies neutrinos at the University of Texas at Arlington, was reading about how neuroscientists watch brain cells think. If Nygren’s brain cells had been lit up with fluorescence while he read, they would have looked like glowing trees branching up into the sky. He had an idea.
To conduct single-molecule fluorescence imaging, scientists release a dye into brain cells—they use rat brain cells—and hit them with light. The dye gets excited and starts to glow. This works because the dye attaches only to certain ions—calcium ions, which act as messengers between neurons.
“It hit me,” Nygren says, “calcium and barium are not that different.”
Just as calcium is important to neuroscientists, barium is important to Nygren. That’s because he is part of the Neutrino Experiment with Xenon TPC, also known as NEXT.
NEXT is searching for proof of a theoretical process called neutrinoless double beta decay. During a double-beta decay, two neutrons within one nucleus transform into two protons, two electrons and two neutrinos. For a double-beta decay to be called “neutrinoless,” the two neutrinos created would need to annihilate one another.
If this happened, scientists would have the answer to an important question in particle physics: Are neutrinos their own antiparticles? If two neutrinos canceled one another, physicists would know that neutrinos and antineutrinos are one and the same.
NEXT looks for neutrinoless double-beta decay in xenon. If xenon went through the process of neutrinoless double-beta decay, its nucleus would transform into barium. Nygren’s neurons were firing because he realized he might be able to use single-molecule fluorescence imaging to search for that new barium nucleus.
Neutrinoless double-beta decay would be an extremely rare process. In a ton of xenon this decay might happen only a few times in a year.
Most radioactive processes happen far more often than neutrinoless double-beta decay. And most materials on Earth include a small amount of naturally occurring radioactive elements. All of this creates a sea of ambient background radiation that scientists must find a way to filter out if they ever want to see evidence of neutrinoless double-beta decay.
Using single-molecule fluorescence imaging could make neutrinoless double-beta decay stand out from the crowd.
“If they succeed in proving the principle of their detector concept, they will eliminate all background except for normal double-beta decay,” says Steven Elliot, scientist at the Majorana Demonstrator at Sanford Underground Research Facility. “This would be a great leap for our field and our understanding of the universe.”
Nygren took his idea to a group of fellow scientists at Arlington.
“We all had no background in biochemistry,” says Nygren’s colleague Ben Jones, an assistant professor. “But we dove into the topic to explore and adapt the technique for our needs.”
The group at Arlington released a calcium-tagging dye in an aqueous environment and found that it was able to grab barium ions and glow. The next step will be to test the technique in a dry environment; they want to eventually employ the dye in a xenon gas chamber.
“We are still at the beginning, but so far the idea to use this technique for our detector looks less crazy every single day,” says Austin McDonald, a research assistant at the University of Texas at Arlington. “We really hope we can realize this in a large-scale project.”
A primer on gravitational-wave detectors
Physicists are searching for gravitational waves all across the spectrum.

Gravitational waves, or ripples in the fabric of space-time, have captured the imagination of physicists since Albert Einstein first predicted them in 1916. But it wasn’t until the 1960s that Joseph Weber, an experimental physicist at the University of Maryland, built the first machine meant to find them.
About 50 years later, scientists finally did it; the Laser Interferometer Gravitational-Wave Observatory detected gravitational waves coming from the merger of two black holes.
The merging black holes LIGO discovered emit gravitational waves at relatively high frequencies. But more massive objects, such as supermassive black holes and merging galaxies, produce waves with longer periods and lower frequencies.
Astronomers are using a wide variety of instruments to seek out gravitational waves at these different frequencies to detect the cosmic events that produce them.
Resonant-mass detectors
Weber’s first gravitational wave detector was a resonant bar detector, or Weber bar. These detectors are big cylindrical metal bars that vibrate at their resonant frequencies when a gravitational wave passes by, a bit like massive tuning forks.
After the many generations of following Weber’s first attempts, most resonant-mass detectors are now out of commission. Physicists use them to search for gravitational waves around the 700- to 3000-hertz region, where they expect to find supernovae, merging neutron stars and possibly even mini black holes. The major limitation of these instruments is that they are sensitive to a very small frequency range.
To increase their chances, some physicists decided to switch from a bar-shaped resonant-mass detector to a spherical one that could detect gravitational waves in all directions and with any polarization, not just some.
One of the most recently built spherical detectors is the Mario Schenberg gravitational-wave detector, which is now at the National Institute for Space Research (INPE) in Brazil. The sphere is around 65 centimeters in diameter and weighs around 1150 kilograms.
This project is still active, though its members are now part of the LIGO collaboration and devote most of their time there.
“We keep going, slowly, but our objective is to make these detectors run perhaps five or 10 years from now,” says Odylio Denys Aguiar, a physicist at INPE and the leader of the project.
Ground-based interferometers
Ground-based interferometers are probably the most well known gravitational-wave detectors, thanks to LIGO’s breakthrough. These detectors have two arms that form the shape of an L. In LIGO’s case, each arm is 4 kilometers long.
In ground-based interferometers, physicists split a laser beam and send it down each arm. The beam bounces off mirrors at each end, travelling back and forth. A passing gravitational wave changes the relative lengths of the arms slightly and shifts the beam’s path, creating a change that physicists can identify.
These observatories can detect short wavelengths, primarily with frequencies in the hundreds of hertz range, making them sensitive to mergers of neutron stars and black holes that are between a few times to tens of times the mass of the sun.
There are a number of ground-based interferometers, both active and under construction. LIGO operates out of twin observatories in Louisiana and Washington state. There are plans to build a third LIGO observatory in India. Virgo and GEO600, which have similar set-ups but shorter arms, are located in Italy and Germany, respectively. KAGRA, an underground interferometer, is under construction in Japan.
These detectors are sensitive to a similar range of frequencies, but there is a key benefit to having many detectors in different parts of the world. Gravitational-wave detectors act like microphones, surveying massive patches of the cosmos from all directions. This increases their chances of finding signs of gravitational waves, but it also makes it difficult to see where exactly they came from. Having more than one detector allows physicists to triangulate a signal to better locate its position on the sky.
Space-based interferometers
Some astronomers plan to bring gravitational-wave astronomy to space. The Laser Interferometer Space Antenna (LISA) has a set-up similar to LIGO, except with three arms over a million kilometers long. Instead of an L-shape, LISA would form an equilateral triangle orbiting the sun, with a satellite placed at each of the vertices. Like in LIGO, a laser beam would go back and forth along the arms, and physicists could detect changes in the length of the arms as a gravitational wave passed through.
The LISA collaboration hopes to launch a space-based observatory around 2034. So far, they have launched the LISA Pathfinder, a short version of one of the arms of the observatory, to test how well it works.
“With the success of LISA Pathfinder, we already know that we can do large parts of the mission,” says Martin Hewitson, a physicist at the Max Planck Institute for Gravitational Physics working on both LISA and LISA Pathfinder. “So there is a lot of scientific and political momentum to make this mission happen earlier.”
In space, the detector will be sensitive to much lower frequencies than the ground-based ones—in LISA’s case, frequencies in the millihertz range. Here, astronomers expect to see gravitational waves from mergers of the supermassive black holes at the center of galaxies. “By looking at these and how they evolve, there is a hope to trace how these galaxies merged and how these black holes have grown over the whole cosmic time,” Hewitson says.
Pulsar timing arrays
Pulsars, spinning neutron stars that constantly emit beams of electromagnetic radiation, are natural timekeepers. Of these, millisecond pulsars are the most regular—to the point that astronomers can predict the time they will arrive on Earth with nanosecond precision.
Physicists use pulsar timing arrays to search for gravitational waves. When a gravitational wave passes, space-time warps between the pulsar and earth. This changes the time of arrival of the pulses, which physicists can then detect with radio telescopes.
“With LIGO, they are trying to detect a deformation much smaller than the diameter of a proton across an instrument that is many kilometers in length—an incredibly tiny signature,” explains Shami Chatterjee, an astronomer at Cornell University working on the North American Nanohertz Observatory for Gravitational Waves (NANOGrav). “For pulsar timing arrays, it's the same scaling—our arms are hundreds or thousands of light years long, but we're trying to measure the same kind of fractional change.”
This technique is sensitive to even lower frequencies than LISA, in the nanohertz range. Here, scientists expect to see a stochastic background of merging supermassive black holes (the sum of all the mergers), binary supermassive black holes, as well as more exotic sources such as cosmic strings and memory bursts, the permanent imprint on space-time left behind by merging supermassive black holes.
There are three major pulsar timing array experiments in operation: NANOGrav, the European Pulsar Timing Array, and the Parkes Pulsar Timing Array in Australia.
Cosmic microwave background detectors
Finally, astronomers are also looking for primordial gravitational waves. These are waves created in the chaos of the very early universe.
One of the ways astronomers do this is quite different from the techniques described above. Rather than watching moving light originating from a laser or a pulsar, they look at a still image of light left over from the time just after the Big Bang—the cosmic microwave background—and try to see evidence of gravitational waves imprinted in it.
“It’s the difference between finding something bobbing up and down in the ocean and taking a snapshot of the ocean and seeing the crests and troughs,” Chatterjee says.
This is extremely difficult because there are many sources of noise, making the feat a bit like finding a specific small ripple in a pool while people are splashing around in it.
Interferometers and pulsar timing arrays are searching for these ancient waves as well. “The primordial gravitational wave background can, in principle, be observed in a very broad range of frequencies, from very low to very high ones,” says Pablo Rosado, an astrophysicist at Monash University studying gravitational wave detection. But according to Rosado, detectors like LIGO might not be able to see this signal because there may be too many binary black holes masking it.
LIGO's discovery was just the beginning. Just as the electromagnetic spectrum spans everything from long radio waves to short gamma rays, the gravitational wave spectrum extends across a huge range of frequencies that require very different instruments to find. Astronomers hope that together, these detectors will find the invisible signals that will help them understand the universe in a whole new light.
99 percent invisible
With a small side project, astronomers discover a new type of galaxy.

In 2011, astronomers Pieter van Dokkum and Roberto “Bob” Abraham found themselves in a restaurant in Toronto nursing something of a mid-life crisis. Abraham, a professor at the University of Toronto, and van Dokkum, at Yale, had become successful scientists, but they discovered that often meant doing less and less science and more and more managing large, complex projects.
“They’re important and they’re great and you feel this tremendous obligation once you’ve reached a certain age to serve on these committees because you have to set things up for the next generation,” Abraham says. “At the same time, it was no longer very much fun.”
The two friends fantasized about finding a small, manageable project that might still have some impact. By the time a few hours had passed, they picked an idea: using new camera lenses to find objects in the sky that emit very little light.
They had no way of knowing then that within the next five years, they’d discover an entirely new class of galactic object.
From the handmade telescopes of Galileo to spacefaring technological marvels like Hubble, all telescopes are designed for one basic task: gathering light. Telescope technology has advanced far enough that Hubble can pick up light from stars that were burning just 400 million years after the universe first popped into existence.
But telescopes often miss objects with light that’s spread out, or diffuse, which astronomers describe as having low surface brightness. Telescopes like Hubble have large mirrors that scatter light from bright objects in the sky, masking anything more diffuse. “There’s this bit of the universe that’s really quite unexplored because our telescope designs are not good at detecting these things,” Abraham says.
When van Dokkum and Abraham sat down at that bar, they decided to try their hands at studying these cosmic castaways. The key turned out to be van Dokkum’s hobby as an amateur insect photographer. He had heard of new camera lenses developed by Canon that were coated with nanoparticles designed to prevent light scattering. Although they were intended for high-contrast photography—say, snapping a photo of a boat in a sunny bay—van Dokkum thought these lenses might be able to spot diffuse objects in the sky.
Abraham was skeptical at first: “Yeah, I’m sure the Canon corporation has come up with a magical optical coating,” he recalls thinking. But when the pair took one to a parking lot in a dark sky preserve in Quebec, they were sold on its capabilities. They acquired more and more lenses—not an easy task, at $12,000 a pop—eventually gathering 48 of them, and arranged them in an ever-growing honeycomb shape to form what can rightly be called a telescope. They named it Dragonfly.
In 2014, both van Dokkum and Abraham were at a conference in Oxford when van Dokkum examined an image that had come in from Dragonfly. (At the time, it had just eight lenses.) It was an image of the Coma Cluster, one of the most photographed galaxy clusters in the universe, and it was dotted with faint smudges that didn’t match any objects in Coma Cluster catalogs.
Van Dokkum realized these smudges were galaxies, and that they were huge, despite their hazy light. They repeated their observations using the Keck telescope, which enabled them to calculate the velocities of the stars inside their mysterious galaxies. One was measured at 50 kilometers per second, 10 times the speed the galaxy should be moving based on the mass of its stars alone.
“We realized that for these extremely tenuous objects to survive as galaxies and not be ripped apart by their movement through space and interactions with other galaxies, there must be much more than meets the eye,” van Dokkum says.
The galaxy, dubbed Dragonfly 44, has less than 1 percent as many stars as the Milky Way, and yet it has to be just as massive. That means that the vast majority of its matter is not the matter that makes up stars and planets and people—everything we can see—but dark matter, which seems to interact with regular matter through gravity alone.
Astronomers have known for decades that galaxies can be made almost entirely of dark matter. But those galaxies were always small, a class known as dwarf galaxies, which have between 100 million and a few billion stars. A dark-matter-dominated galaxy as large as the Milky Way, with its 200 billion or more stars, needed an entirely new category. Van Dokkum and Abraham coined a term for them: ultradiffuse.
“You look at a galaxy and you see this beautiful spiral structure and they’re gorgeous. I love galaxies,” Abraham says. “But what you see is really just kind of the frosting on the cake. The cake is the dark matter.”
No one knows how many of these galaxies might exist, or whether they can have an even larger percentage of dark matter than Dragonfly 44. Perhaps there are galaxies that have no luminous matter at all, simply massive dark blobs hurtling through empty space. Though such galaxies have thus far evaded observation, evidence of their existence may be lurking in unexamined data from the past.
And Dragonfly could be the key for finding them. “When people knew they were real and that these things could exist and could be part of these galaxy clusters, suddenly they turned up in large numbers,” van Dokkum says. “They just escaped attention for all these decades.”
It came from the physics lab
Settle in for a physics-themed Halloween movie marathon.
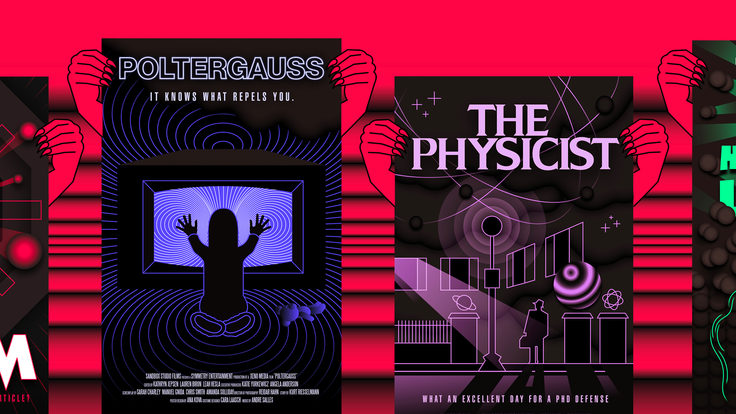
Looking for a way to celebrate Halloween? Has 2016 got you too spooked to go outside? Pop some corn and sample Symmetry’s little-known series of physics horror films instead. (Actual movies not included.)
Someone’s taken their love of the Higgs boson one step too far!
I need an old theorist and a young theorist.
Entropy’s coming to get you, Barbara!
He’s heeere.
Citizen scientists join search for gravitational waves
A new project pairs volunteers and machine learning to sort through data from LIGO.

Barbara Téglás was looking to try something different while on a break from her biotechnology work.
So she joined Zooniverse, a website dedicated to citizen science projects, and began to hunt pulsars and classify cyclones from her home computer.
“It’s a great thing that scientists share data and others can analyze it and participate,” Téglás says. “The project helps me stay connected with science in other fields, from anywhere.”
In April, at her home in the Caribbean Islands, Téglás saw a request for volunteers to help with a new gravitational-wave project called Gravity Spy. Inspired by the discovery of gravitational waves by the Laser Interferometer Gravitational-wave Observatory, or LIGO, she signed up the same day.
“To be a complete outsider and have the opportunity to contribute to an astrophysics project such as LIGO, it’s extraordinary,” Téglás says.
Tuning out the noise
It took a century after Albert Einstein predicted the existence of gravitational waves—or ripples in space-time—for scientists to build an instrument sophisticated enough to see them. LIGO observed these ripples for the first (and second) time, using two L-shaped detectors called interferometers designed to measure infinitesimal changes in distance. These changes were generated by two black holes that collided a billion years in the past, giving off gravitational waves that eventually passed through Earth. As they traveled through our planet, these gravitational waves stretched and shrank the 4-kilometer arms of the detectors.
The LIGO detectors can measure a change in distance about 10,000 times smaller than the diameter of a proton. Because the instruments are so sensitive, this also makes them prone to capturing other vibrations, such as earthquakes or heavy vehicles driving near the detectors. Equipment fluctuations can also create noise.
The noise, also called a glitch, can move the arms of the detector and potentially mimic an astrophysical signal.
The two detectors are located nearly 2000 miles apart, one in Louisiana and the other in Washington state. Gravitational waves from astrophysical events will hit both detectors at nearly the same time, since gravitational waves travel straight through Earth at the speed of light. However, the distance between the two makes it unlikely that other types of vibrations will be felt simultaneously.
“But that’s really not enough,” says Mike Zevin, a physics and astronomy graduate student at Northwestern University and a member of the Gravity Spy science team. “Glitches happen often enough that similar vibrations can appear in both detectors at nearly the same time. The glitches can tarnish the data and make it unusable.”
Gravity Spy enlists the help of volunteers to analyze noise that appears in LIGO detectors.
This information is converted to an image called spectrogram, and the patterns show the time and frequencies of the noise. Shifts in blue, green and yellow indicate the loudness of the glitch, or how much the noise moved the arms of the detector. The glitches show up frequently in the large amount of information generated by the detectors.
“Some of these glitches in the spectrograms are easily identified by computers, while others aren’t,” Zevin says. “Humans are actually better at spotting new patterns in the images.”
The Gravity Spy volunteers are tasked with labeling these hard-to-identify categories of glitches. In addition, the information is used to create training sets for computer algorithms.
As the training sets grow larger, the computers become better at classifying glitches. That can help scientists eliminate the noise from the detectors or find ways to account for glitches as they look at the data.
“One of our goals is to create a new way of doing citizen science that scales with the big-data era we live in now,” Zevin says.
Gravity Spy is a collaboration between Adler Planetarium, California State University-Fullerton, Northwestern University, Syracuse University, University of Alabama at Huntsville, and Zooniverse. The project is supported by an interdisciplinary grant from the National Science Foundation.
About 1400 people volunteered for initial tests of Gravity Spy. Once the beta testing of Gravity Spy is complete, the volunteers will look at new images created when LIGO begins to collect data during its second observing run.
A human endeavor
The project also provides an avenue for human-computer interaction research.
Another goal for Gravity Spy is to learn the best ways to keep citizen scientists motivated while looking at immense data sets, says Carsten Oesterlund, information studies professor at Syracuse University and member of the Gravity Spy research team.
“What is really exciting from our perspective is that we can look at how human learning and machine learning can go hand-in-hand,” Oesterlund says. “While the humans are training the machines, how can we organize the task to also facilitate human learning? We don’t want them simply looking at image after image. We want developmental opportunities for the volunteers.”
The researchers are examining how to encourage the citizen scientists to collaborate as a team. They also want to support new discoveries, or make it easier for people to find unique sets of glitches.
One test involves incentives—in an earlier study, the computing researchers found if a volunteer knows that they are the first to classify an image, they go on to classify more images.
“We’ve found that the sense of novelty is actually quite motivating,” says Kevin Crowston, a member of the Gravity Spy science team and associate dean for research at Syracuse University’s School of Information Studies.
Almost every day, Téglás works on the Gravity Spy project. When she has spare time, she sits down at her computer and looks at glitches. Since April, she’s classified nearly 15,000 glitches and assisted other volunteers with hundreds of additional images through talk forums on Zooniverse.
She’s pleased that her professional skills developed while inspecting genetics data can also help many citizen science projects.
On her first day with Gravity Spy, Téglás helped identify a new type of glitch. Later, she classified another unique glitch called “paired doves” after its repeating, chirp-like patterns, which closely mimic the signal created by binary black holes. She’s also found several new variations of known glitches. Her work is recognized in LIGO’s log, and the newly found glitches are now part of the official workflow for the experiment.
Different experiences, backgrounds and ways of thinking can make citizen science projects stronger, she says.
“For this project, you’re not only using your eyes,” Téglás says. “It’s also an opportunity to understand an important experiment in modern science.”
Recruiting team geoneutrino
Physicists and geologists are forming a new partnership to study particles from inside the planet.

The Earth is like a hybrid car.
Deep under its surface, it has two major fuel tanks. One is powered by dissipating primordial energy left over from the planet’s formation. The other is powered by the heat that comes from radioactive decay.
We have only a shaky understanding of these heat sources, says William McDonough, a geologist at the University of Maryland. “We don’t have a fuel gauge on either one of them. So we’re trying to unravel that.”
One way to do it is to study geoneutrinos, a byproduct of the process that burns Earth’s fuel. Neutrinos rarely interact with other matter, so these particles can travel straight from within the Earth to its surface and beyond.
Geoneutrinos hold clues as to how much radioactive material the Earth contains. Knowing that could lead to insights about how our planet formed and its modern-day dynamics. In addition, the heat from radioactive decay plays a key role in driving plate tectonics. Understanding the composition of the planet and the motion of the plates could help geologists model seismic activity.
To effectively study geoneutrinos, scientists need knowledge both of elementary particles and of the Earth itself. The problem, McDonough says, is that very few geologists understand particle physics, and very few particle physicists understand geology. That’s why physicists and geologists have begun coming together to build an interdisciplinary community.
“There’s really a need for a beyond-superficial understanding of the physics for the geologists and likewise a nonsuperficial understanding of the Earth by the physicists,” McDonough says, “and the more that we talk to each other, the better off we are.”
There are hurdles to overcome in order to get to that conversation, says Livia Ludhova, a neutrino physicist and geologist affiliated with Forschungzentrum Jülich and RWTH Aachen University in Germany. “I think the biggest challenge is to make a common dictionary and common understanding—to get a common language. At the basic level, there are questions on each side which can appear very naïve.”
In July, McDonough and Gianpaolo Bellini, emeritus scientist of the Italian National Institute of Nuclear Physics and retired physics professor at the University of Milan, led a summer institute for geology and physics graduate students to bridge the divide.
“In general, geology is more descriptive,” he says. “Physics is more structured.”
This can be especially troublesome when it comes to numerical results, since most geologists are not used to working with the defined errors that are so important in particle physics.
At the summer institute, students began with a sort of remedial “preschool,” in which geologists were taught how to interpret physical uncertainty and the basics of elementary particles and physicists were taught about Earth’s interior. Once they gained basic knowledge of one another’s fields, the scientists could begin to work together.
This is far from the first interdisciplinary community within science or even particle physics. Ludhova likens it to the field of radiology: There is one expert to take an X-ray and another to determine a plan of action once all the information is clear. Similarly, particle physicists know how to take the necessary measurements, and geologists know what kinds of questions they could answer about our planet.
Right now, only two major experiments are looking for geoneutrinos: KamLAND at the Kamioka Observatory in Japan and Borexino at the Gran Sasso National Laboratory in Italy. Between the two of them, these observatories detect fewer than 20 geoneutrinos a year.
Because of the limited results, geoneutrino physics is by necessity a small discipline: According to McDonough, there are only about 25 active neutrino researchers with a deep knowledge of both geology and physics..
Over the next decade, though, several more neutrino detectors are anticipated, some of which will be much larger than KamLAND or Borexino. The Jiangmen Underground Neutrino Observatory (JUNO) in China, for example, should be ready in 2020. Whereas Borexino’s detector is made up of 300 tons of active material, and KamLAND’s contains 1000, JUNO’s will have 20,000 tons.
The influx of data over the next decade will allow the community to emerge into the larger scientific scene, Bellini says. “There are some people who say ‘now this is a new era of science’—I think that is exaggerated. But I do think that we have opened a new chapter of science in which we use the methods of particle physics to study the Earth.”
Hunting the nearly un-huntable
The MINOS and Daya Bay experiments weigh in on the search for sterile neutrinos.

In the 1990s, the Liquid Scintillator Neutrino Detector (LSND) experiment at Los Alamos National Laboratory saw intriguing hints of an undiscovered type of particle, one that (as of yet) cannot be detected. In 2007, the MiniBooNE experiment at the US Department of Energy’s Fermi National Accelerator Laboratory followed up and found a similar anomaly.
Today scientists on two more neutrino experiments—the MINOS experiment at Fermilab and the Daya Bay experiment in China—entered the discussion, presenting results that limit the places where these particles, called sterile neutrinos, might be hiding.
“This combined result was a two-year effort between our collaborations,” says MINOS scientist Justin Evans of the University of Manchester. “Together we’ve set what we believe is a very strong limit on a very intriguing possibility.”
In three separate papers—two published individually by MINOS and Daya Bay and one jointly, all in Physical Review Letters—scientists on the two experiments detail the results of their hunt for sterile neutrinos.
Both experiments are designed to see evidence of neutrinos changing, or oscillating, from one type to another. Scientists have so far observed three types of neutrinos, and have detected them changing between those three types, a discovery that was awarded the 2015 Nobel Prize in physics.
What the LSND and MiniBooNE experiments saw—an excess of electron neutrino-like signals—could be explained by a two-step change: muon neutrinos morphing into sterile neutrinos, then into electron neutrinos. MINOS and Daya Bay measured the rate of these steps using different techniques.
MINOS, which is fed by Fermilab’s neutrino beam—the most powerful in the world—looks for the disappearance of muon neutrinos. MINOS can also calculate how often muon neutrinos should transform into the other two known types and can infer from that how often they could be changing into a fourth type that can’t be observed by the MINOS detector.
Daya Bay performed a similar observation with electron anti-neutrinos (assumed, for the purposes of this study, to behave in the same way as electron neutrinos).
The combination of the two experiments’ data (and calculations based thereon) cannot account for the apparent excess of neutrino-like signals observed by LSND. That along with a reanalysis of results from Bugey, an older experiment in France, leaves only a very small region where sterile neutrinos related to the LSND anomaly could be hiding, according to scientists on both projects.
“There’s a very small parameter space left that the LSND signal could correspond to,” says Alex Sousa of the University of Cincinnati, one of the MINOS scientists who worked on this result. “We can’t say that these light sterile neutrinos don’t exist, but the space where we might find them oscillating into the neutrinos we know is getting narrower.”
Both Daya Bay and MINOS’ successor experiment, MINOS+, have already taken more data than was used in the analysis here. MINOS+ has completely analyzed only half of its collected data to date, and Daya Bay plans to quadruple its current data set. The potential reach of the final joint effort, says Kam-Biu Luk, co-spokesperson of the Daya Bay experiment, “could be pretty definitive.”
The IceCube collaboration, which measures atmospheric neutrinos with a detector deep under the Antarctic ice, recently conducted a similar search for sterile neutrinos and also came up empty.
All of this might seem like bad news for fans of sterile neutrinos, but according to theorist André de Gouvea of Northwestern University, the hypothesis is still alive.
Sterile neutrinos are “still the best new physics explanation for the LSND anomaly that we can probe, even though that explanation doesn’t work very well,” de Gouvea says. “The important thing to remember is that these results from MINOS, Daya Bay, Ice Cube and others don’t rule out the concept of sterile neutrinos, as they may be found elsewhere.”
Theorists have predicted the existence of sterile neutrinos based on anomalous results from several different experiments. The results from MINOS and Daya Bay address the sterile neutrinos predicted based on the LSND and MiniBooNE anomalies. Theorists predict other types of sterile neutrinos to explain anomalies in reactor experiments and in experiments using the chemical gallium. Much more massive types of sterile neutrinos would help explain why the neutrinos we know are so very light and how the universe came to be filled with more matter than antimatter.
Searches for sterile neutrinos have focused on the LSND neutrino excess, de Gouvea says, because it provides a place to look. If that particular anomaly is ruled out as a key to finding these nigh-undetectable particles, then they could be hiding almost anywhere, leaving no clues. “Even if sterile neutrinos do not explain the LSND anomaly, their existence is still a logical possibility, and looking for them is always interesting,” de Gouvea says.
Scientists around the world are preparing to search for sterile neutrinos in different ways.
Fermilab is preparing a three-detector suite of short-baseline experiments dedicated to nailing down the cause of both the LSND anomaly and an excess of electrons seen in the MiniBooNE experiment. These liquid-argon detectors will search for the appearance of electron neutrinos, a method de Gouvea says is a more direct way of addressing the LSND anomaly. One of those detetors, MicroBooNE, is specifically chasing down the MiniBooNE excess.
Scientists at Oak Ridge National Laboratory are preparing the Precision Oscillation and Spectrum Experiment (PROSPECT), which will search for sterile neutrinos generated by a nuclear reactor. CERN’s SHiP experiment, which stands for Search for Hidden Particles, is expected to look for sterile neutrinos with much higher predicted masses.
Obtaining a definitive answer to the sterile neutrino question is important, Evans says, because the existence (or non-existence) of these particles might impact how scientists interpret the data collected in other neutrino experiments, including Japan’s T2K, the United States’ NOvA, the forthcoming DUNE, and other future projects. DUNE in particular will be able to look for sterile neutrinos across a broad spectrum, and evidence of a fourth kind of neutrino would enhance its already rich scientific program.
“It’s absolutely vital that we get this question resolved,” Evans says. “Whichever way it goes, it will be a crucial part of neutrino experiments in the future.”